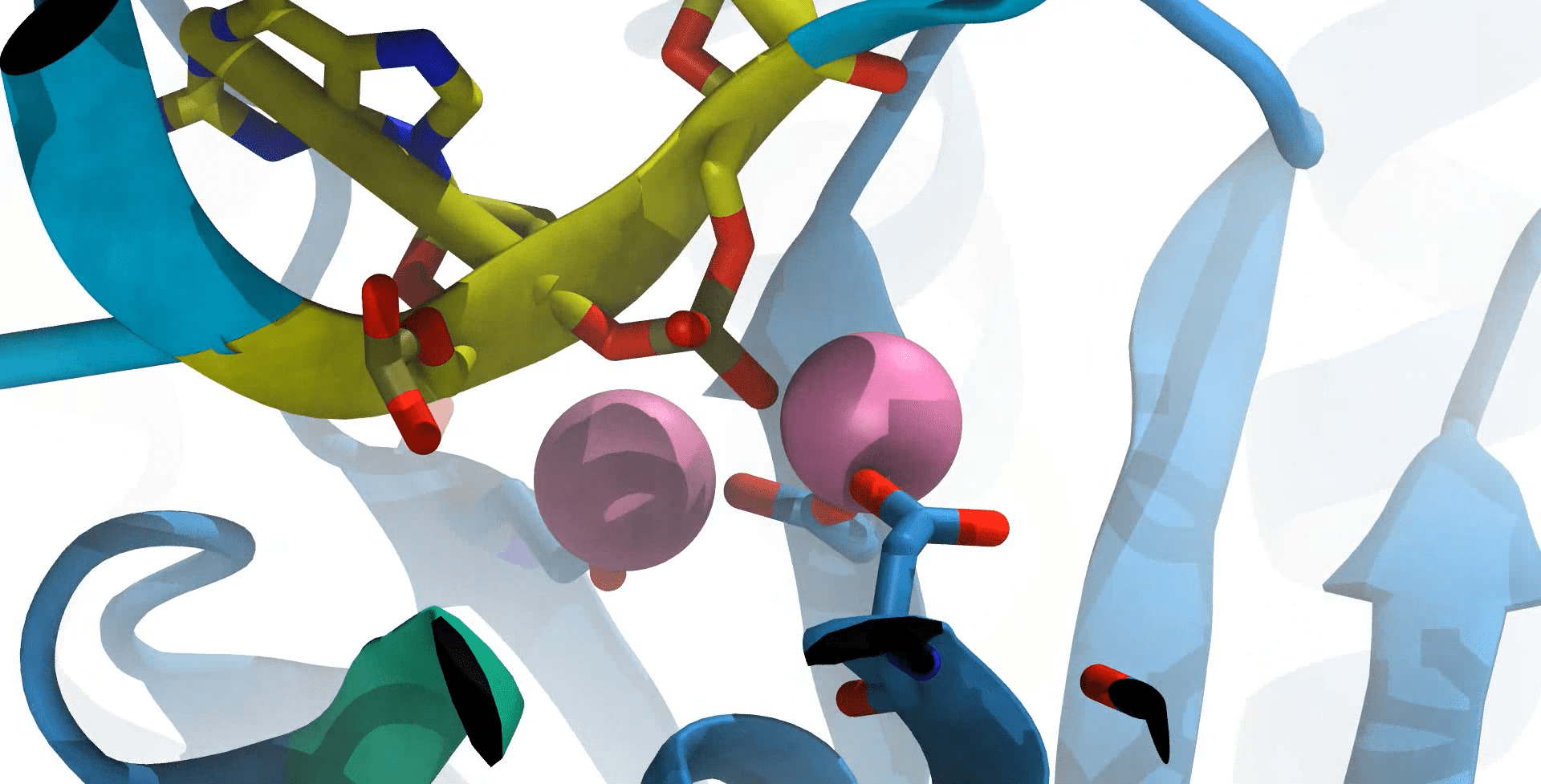
Scientists used the second-generation DESRES Anton supercomputer hosted at PSC to show how a critical protein for furture gene therapy pauses before completing its cutting of a target DNA. Credit: Aakash Saha
Understanding Unexpected Motion of Protein Will Be Crucial for Engineering it for DNA Detection, Editing
The discovery of the CRISPR-Cas9 system revolutionized genetic therapy because of its precision and programmability. A relative of the Cas9 protein, Cas12a, can make the CRISPR-Cas system even more precise. But there’s a pause in the Cas12a protein’s function of clipping DNA strands that limits its usefulness. Using the Anton supercomputer developed by D. E. Shaw Research (DESRES) and hosted at PSC, a team led from the University of California, Riverside (UC Riverside), has revealed an unexpected movement of the protein that explains the delay. This information will be critical for engineering Cas12a for future genetic therapies and diagnostic tests.
WHY IT’S IMPORTANT
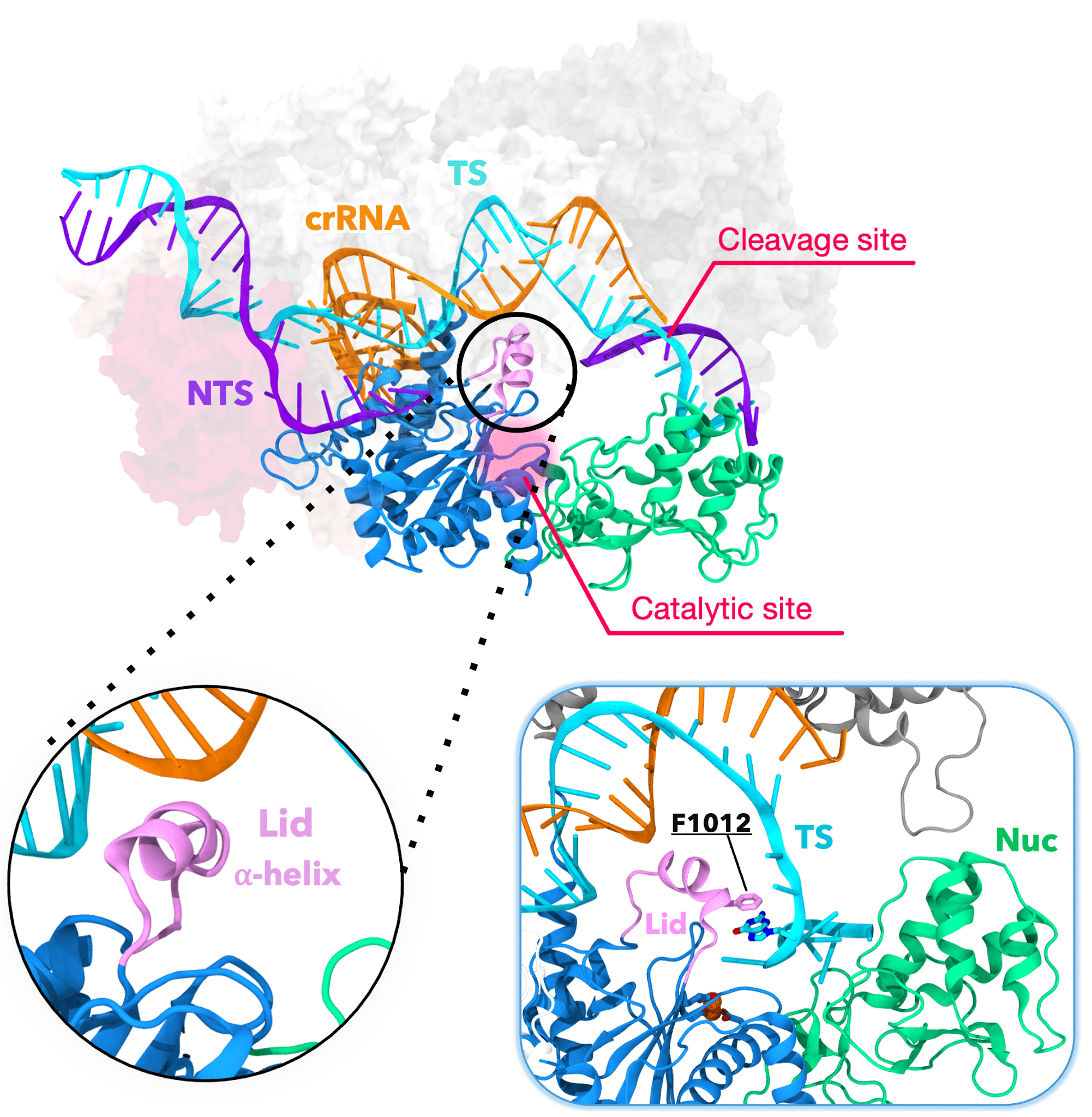
The CRISPR-Cas12a protein showing the alpha helical lid that guides (inset, right) the target DNA strand (TS) to the catalytic site for cleavage. Credit: Aakash Saha
The CRISPR-Cas system evolved in bacteria to cut invading virus DNA. Scientists have made use of its abilities to develop tests to rapidly detect the SARS-CoV-2 virus, or any other diseases with a genetic marker. Even more ambitiously, doctors would like to use CRISPR-Cas to edit faulty genes, curing genetic disorders by correcting the DNA sequences that cause them.
One type of CRISPR-Cas protein, Cas9, has made a lot of headlines for its ability to edit genes with precision. The related Cas12a protein, though, has some advantages over Cas9 that scientists are only beginning to leverage.
Cas12a is more specific than Cas9, making it attractive for gene editing. It also has an interesting property: once it cuts DNA matching its nucleic acid template, its active site remains open and activated, and able to cut just about any DNA. Scientists realized that, when given a target DNA sequence contained in a particular gene of interest, the protein will cut that DNA first if it’s present in a sample — say, from a swabbed surface — and will then cut a reporter DNA that fluoresces once it’s cut. While only a tiny amount of the target gene may be present, by having the test tube filled with reporter DNA, you can nevertheless get a strong, glowing signal that the gene is there.
Scientists understand at a certain level of detail how and why this pause happens. DNA is usually double-stranded, with each strand matching the other. While the protein cuts the DNA strand opposite of its target sequence quickly, it then pauses before cutting the strand containing the target sequence. This makes the protein relatively slow compared with its Cas9 cousin — and that’s a problem for applying Cas12a to other gene-detection and therapy goals.
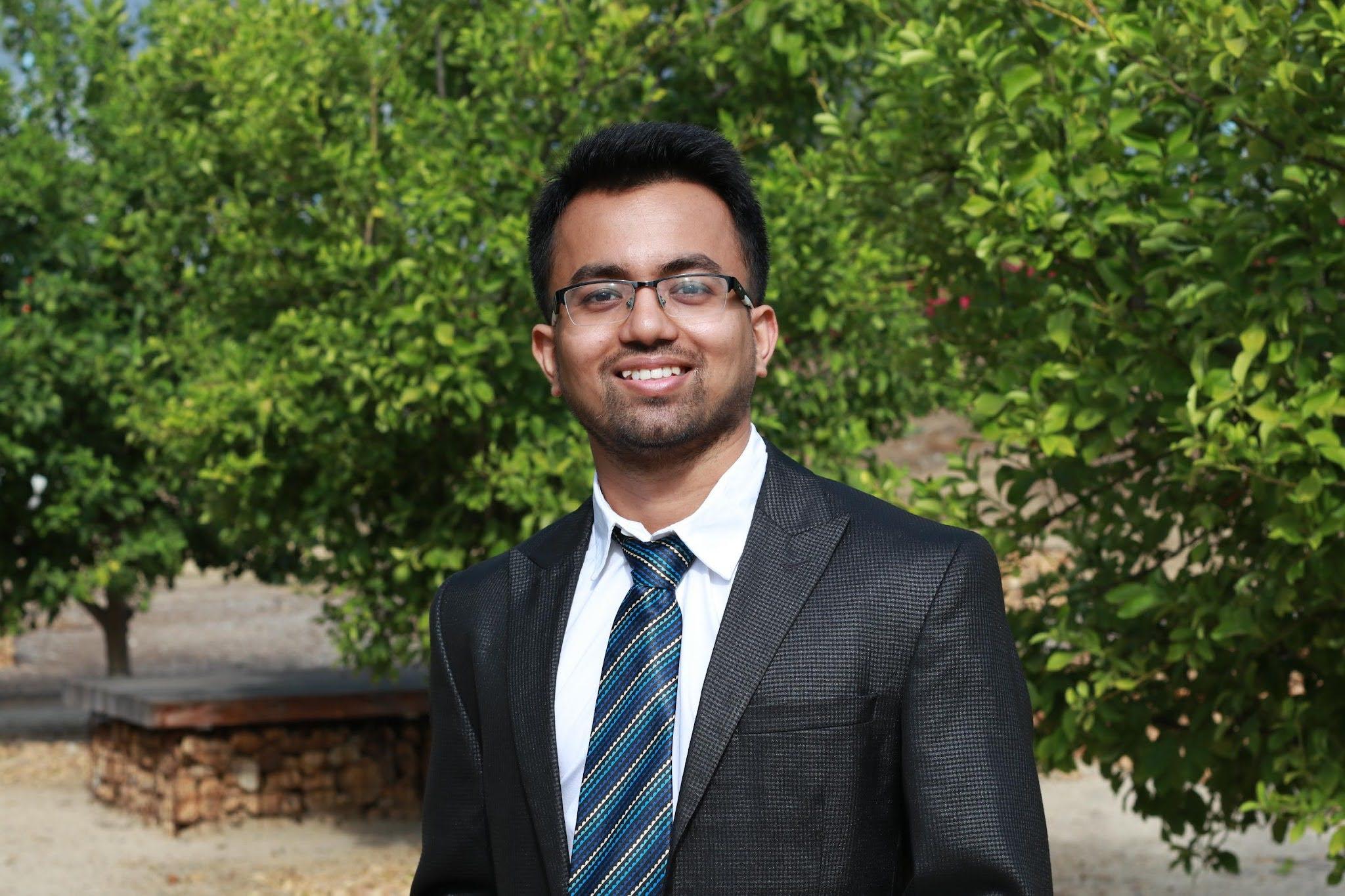
“There is a certain amount of delay in [Cas12a] cleaving one of the DNA strands, and that is what we [were trying] to address. Why is it happening? Who is responsible? Because it is extremely important to have the foundational science laid out … Only then can you advance with engineering strategies to change and expedite.” — Aakash Saha, UC Riverside
To investigate this problem, then-graduate-student Aakash Saha collaborated with Mohd Ahsan and Pablo R. Arantes, postdoctoral fellows in the laboratory of Giulia Palermo, an associate professor at the UC Riverside. They wanted to better understand how the DNA strand in Cas12a moves towards the active site to accomplish the protein’s DNA-cutting dance. In particular, the team wanted to figure out why, in a much more detailed sense, the enzyme pauses. This basic knowledge could potentially allow scientists to tailor the protein to carry out its functions in a way suited to various detection and disease-treatment tasks.
The Palermo team dissected the movements of Cas12a that lead to double-stranded DNA cleavages using Anton, a special-purpose supercomputer for molecular dynamics simulation that was designed and constructed by DESRES. A second-generation Anton machine is made available without cost by DESRES, and is hosted at PSC with operational funding support by the National Institutes of Health.
HOW PSC HELPED
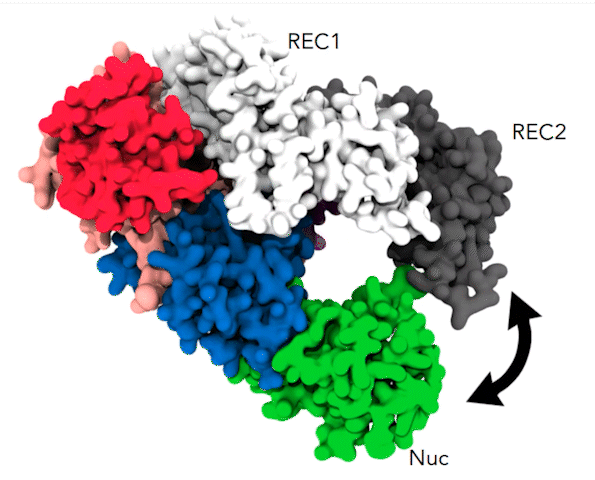
The dynamic alpha-helical “lid” and the coordinated movements between REC1 (white), REC2 (gray), and Nuc (green) cause the parts of the Cas12a protein to pinch together, a movement that brings the target DNA strand to the protein’s active site for cleavage. Credit: Aakash Saha
Previous studies of Cas12a include X-ray and cryo-electron microscope structures, laboratory experiments monitoring its behavior, and simulations on general-purpose supercomputers. These suggested that the protein first matches the target DNA strand to a short segment of template DNA contained in the protein. This happens in the protein’s active site, a kind of pocket that contains components that carry out the DNA recognition and cutting.
While one part of the active site is holding onto the target DNA, another part cuts the strand of DNA opposite the target — the non-target strand. Because the target strand is some distance from the active site, it needs to be chaperoned in order to be cut. Scientists reasoned that the movement of the DNA likely caused the delay in the second cut. But no one knew how it happened.
The second-generation Anton supercomputer at PSC can simulate large numbers of atoms roughly 10 times faster than general-purpose systems. This gives it the ability to simulate molecules for microseconds in a reasonable amount of real time, revealing rare motions that nevertheless are pivotal for the biomolecules’ functions.
“When we ran the 10-microsecond simulation, we saw it at the 6- to 7-microsecond time point … We were just open-mouthed, saying, oh, my God! This is actually a new finding … If I were to do this same simulation [with general-purpose supercomputers] for a CRISPR system with the nucleic acid and the water [surrounding the protein], it would have taken almost four and a half months. But [with Anton] we achieve this in a matter of an hour.” — Aakash Saha, UC Riverside
By simulating 10 microseconds at a time, Anton revealed something that earlier, shorter simulations hadn’t been able to capture. At a time point of 6 to 7 microseconds, a helix-shaped “lid” in the active site that had up until that point held the target DNA in place began to change position. It guided the DNA toward the part of the active site that performed the cutting. Along with tightly coordinated bending elsewhere in the protein, this put the target DNA in position to be snipped. These highly orchestrated movements allowed Cas12a to cut both DNA strands and were the cause of the delay in the second cut.
Alongside this work, the UC Riverside scientists’ colleagues in Zürich carried out real-world lab experiments that confirmed the simulated findings. Together with further simulations showing that these large movements in the active site only happened when cutting DNA and not at other times, this gave the collaborators confidence that the simulations were reproducing Cas12a’s real-world behavior. The team reported their results in the prestigious journal Nature Communications in February 2024.